- Journal List
- HHS Author Manuscripts
- PMC1890044

Molecular Mechanisms of Cerebrospinal Fluid Production
Abstract
The epithelial cells of the choroid plexuses secrete cerebrospinal fluid (CSF), by a process which involves the transport of Na+, Cl- and HCO3- from the blood to the ventricles of the brain. The unidirectional transport of ions is achieved due to the polarity of the epithelium, i.e. the ion transport proteins in the blood-facing (basolateral) membrane are different to those in the ventricular (apical) membrane. The movement of ions creates an osmotic gradient which drives the secretion of H2 O. A variety of methods (e.g. isotope flux studies, electrophysiological, RT-PCR, in situ hybridization and immunocytochemistry) have been used to determine the expression of ion transporters and channels in the choroid plexus epithelium. Most of these transporters have now been localized to specific membranes. For example, Na+-K+ ATPase, K+ channels and Na+-2Cl--K+ cotransporters are expressed in the apical membrane. By contrast the basolateral membrane contains Cl--HCO3 exchangers, a variety of Na+ coupled HCO3- transporters and K+-Cl- cotransporters. Aquaporin 1 mediates water transport at the apical membrane, but the route across the basolateral membrane is unknown. A model of CSF secretion by the mammalian choroid plexus is proposed which accommodates these proteins. The model also explains the mechanisms by which K+ is transported from the CSF to the blood.
The cerebrospinal fluid (CSF) is a major part of the extracellular fluid of the CNS. The CSF fills the ventricles of the brain, the spinal canal and the subarachnoid space (Fig. 1), and in humans has a total volume of approximately 140 ml. The CSF is separated from neuronal tissue by the ependyma (which lines the ventricles and canals), and the pia (which covers the external surface of the brain). The composition of the CSF does, however, influence neuronal activity, notably in the central chemoreceptors of the medulla oblongata which control respiration by responding to changes in CSF pH.
The locations of the choroid plexuses and the distribution of CSF in the human brain. The CSF is shown as the stippled area and the choroid plexuses are shown as the solid black structures.
The CSF has a number of important functions. It helps provide mechanical support for the brain, i.e. the brain “floats” in the CSF reducing its effective weight by more than 60% (Segal, 1993). CSF also acts as a drainage pathway for the brain, by providing a “sink” into which products of metabolism or synaptic activity are diluted and subsequently removed (Segal, 1993). The CSF may also be an important route by which some nutrients reach the CNS (Johanson, 1999). A final putative role for the CSF is that it acts as a route of communication within the CNS, i.e. it carries hormones and transmitters between different areas of the brain (Johanson, 1999).
The compositions of plasma and CSF are very similar (Table 1), with the only major difference being protein, which has a greatly reduced concentration in the CSF. The CSF is not, however, an ultrafiltrate of the plasma but is actively secreted by the choroid plexuses (Davson et al., 1987). This was clearly demonstrated in experiments showing that the concentrations of some ions in the CSF are very carefully regulated, and more importantly are independent of variations in the plasma concentrations of these ions, e.g. K+ (Husted and Reed, 1976; Jones and Keep, 1987), HCO3- (Husted and Reed, 1977) and Ca2+ (Murphy et al., 1986). The composition of an ultrafiltrate could not be regulated in this manner.
Table 1
The composition of the CSF and plasmaa
Plasma | CSF | |
---|---|---|
Na+(mM) | 155 | 151 |
K+(mM) | 4.6 | 3.0 |
Mg2+(mM) | 0.7 | 1.0 |
Ca2+(mM) | 2.9 | 1.4 |
Cl-(mM) | 121 | 133 |
HCO3-(mM) | 26.2 | 25.8 |
Glucose (mM) | 6.3 | 4.2 |
Amino acids (mM) | 2.3 | 0.8 |
pH | 7.4 | 7.4 |
Osmolality (mosmol.Kg H2O-1) | 300 | 305 |
*Protein (mg 100 g-1) | 6500 | 25 |
The concentrations of the components in bold type are regulated by the choroid plexuses.
The CSF is constantly produced, and in humans the total volume is replaced about four times each day. Thus, the total amount of CSF produced in 24 h is about 600 ml (Wright, 1978). The majority of this CSF is produced by the four choroid plexuses (one in each ventricle of the brain; see Fig. 1). In humans the choroid plexuses weigh about 2 g in total so that the rate of CSF secretion is approximately 0.2 ml min-1 per g of tissue. This rate of secretion is significantly higher than in many other secretory epithelia, e.g. guinea-pig pancreas=0.06 ml min-1 per g tissue. The aim of this review is to examine the mechanism by which CSF is secreted.
The choroid plexuses
The choroid plexuses are branched structures made up of numerous villi which project into the ventricles of the brain. Each villus is composed of a single layer of epithelial cells overlying a core of connective tissue and blood capillaries (Fig. 2A). The choroid plexuses are highly vascularized leading to a very good supply of blood, e.g. the rat lateral and fourth ventricle plexuses receive between three and 4 ml min-1 per g of tissue (Szmydynger-Chodobska et al., 1994). This is almost 10 times greater than the flow to the cerebral cortex. The capillaries in the choroid plexus, unlike those in the majority of the cerebral circulation, are fenestrated and hence provide little resistance to the movement of small molecules, ions and water (Segal, 1993). A barrier is formed, however, by the junctional complexes between the epithelial cells, which restrict the passage of molecules and ions into the CSF (Fig. 2C). The choroid plexus epithelial cells therefore form what is known as the blood-CSF barrier.
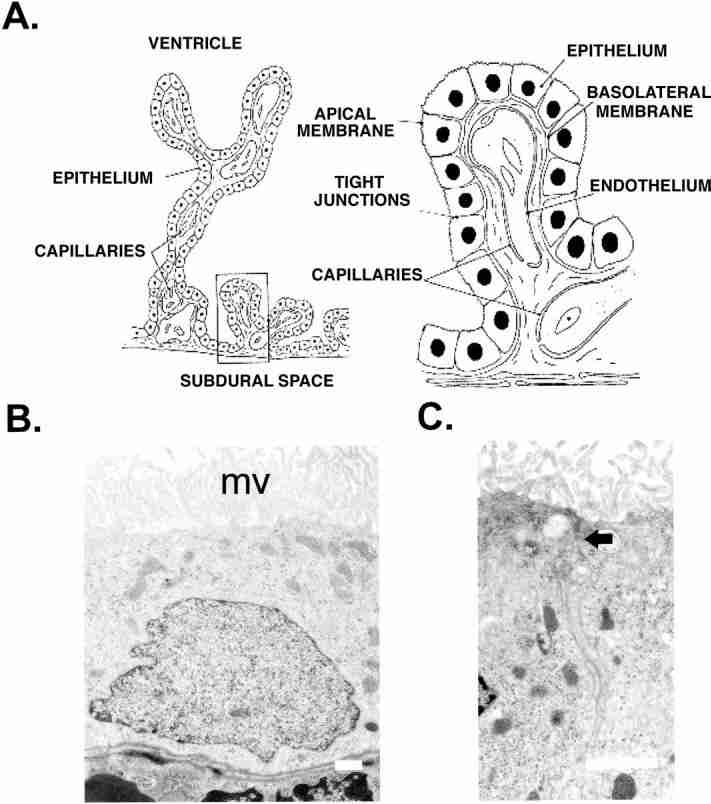
The microscopic structure of the choroid plexus. (A) Branched structure of the choroid plexus with villi projecting into the ventricle of the brain. Each plexus consists of a network of capillaries covered by a single layer of cuboidal epithelial cells. (B) Electron micrograph of choroid plexus epithelial cells from the rat fourth ventricle. The apical membrane has many well-developed microvilli (mv), and the basolateral membrane displays multiple infoldings. Scale bar=1 μm. (C) Junctional complex linking epithelial cells. The arrow shows the position of the zona occludens and the scale bar=1 μm.
In addition to a barrier function, the choroid plexus epithelium is also superbly adapted for secretion. The electron micrograph (Fig. 2B) shows the ultrastructure of rat choroid plexus epithelial cells. They have a very well-developed brush border (apical) membrane which faces into the ventricle. The basolateral membrane on the blood side of the epithelium also displays a number of infoldings which increase the surface area of this membrane. In addition the epithelial cells contain many mitochondria and have well-developed endoplasmic reticulum (Fig. 2B). These are typical features of secretory cells.
Epithelial cell polarity and secretion
To understand the process of CSF secretion it is perhaps useful to first consider the mechanism of fluid secretion by other epithelia. Fluid secretion in all epithelia has been found to be dependent on the unidirectional transport of ions, which creates an osmotic gradient driving the movement of water. Unidirectional transport of ions (either secretion or absorption) can be achieved due to the polarised expression of membrane transport proteins in the apical and basolateral membranes of the epithelial cells (Steward and Case, 1989). In most epithelia secretion is dependent on the transport of Cl-, which is accumulated in the epithelial cells by transporters in the basolateral membrane. Cl- can then leave the epithelial cells via ion channels in the apical membrane (Steward and Case, 1989). The presence of Cl- on the apical side of the epithelium generates an electrical gradient for the transport of Na+ via the paracellular pathway, i.e. through the junctional complexes between the cells. Water moves via either aquaporin water channels in the cell membranes or the paracellular pathway (Steward and Case, 1989).
The choroid plexuses secrete Na+ and Cl-, but HCO3- is also secreted (Wright, 1978). Furthermore the whole secretory process is highly dependent on HCO3- (Saito and Wright, 1983, 1984). The epithelium of the choroid plexus also mediates the net absorption of K+ from the CSF to the blood (Wright, 1978). The transport of K+ is important in maintaining the [K+] in the CSF, but mechanistically it is closely linked to the process of CSF secretion. (It is important to remember that there are net fluxes of other ions, e.g. Ca2+, organic anions and organic cations, across the choroid plexus. These are vital for the normal function of the CNS; however, they are not discussed in this article because they do contribute significantly to the osmotic gradient which drives CSF secretion.) This review will discuss the mechanism of HCO3-, Na+, Cl- and H2 O secretion, and the absorption of K+ by the choroid plexus epithelium.
Ion transport protein expression in the choroid plexus
Until the late 1980s our knowledge of ion transport in the choroid plexuses was based largely on the results of radioisotope flux studies in intact tissue preparations, and of experiments which examined the effects of transport inhibitors on the rate of CSF production. These studies produced many significant data (for reviews see Brown and Garner, 1993; Segal, 1993; Johanson, 1999; Speake et al., 2001) however, the results were often confusing and sometimes even contradictory. Problems arose in the interpretation of such data because of the lack of specificity of the transport inhibitors employed, and because it was difficult to determine the polarity of transporter expression using these methods.
In the last 15 years, the application of molecular biological methods such as RT-PCR, in situ hybridization and immunocytochemistry has enabled the more precise determination of transporter expression. Furthermore, patch clamp electrophysiological methods have been used to characterize the major ion conductances in mammalian choroid plexus cells. The following sections provide details on the molecular identity, localization and properties of the ion transport proteins identified to date in mammalian choroid plexus epithelial cells. Many of the data on transporter expression are summarized in Table 2. The transporters are generally referred to by their common, descriptive names. The HUGO (Human Genome Organization) name of each transporter, however, is also given in the text and Table 2.
Table 2
Molecular identities of the ion transporters and channels expressed in mammalian choroid plexus
Common name | Isoform | HUGO | mRNA protein | Mem. | References |
---|---|---|---|---|---|
Na+-K+ATPase | α1 | ATP1A1 | m,p | A | Masuzawa et al., 1984; Ernst et al., al.,1986; Watts et al., 1991; Zlokovic et 1993 |
βb | ATP1B1 | m,p | A | ||
β2 | ATP1B2 | m,p | A | ||
Na+-2Cl- -K+ cotransporter | NKCC1 | SLC12A2 | m,p | A | Plotkin et al., 1997; Wu et al., 1998 |
K+-Cl- cotransporters | KCC1 | SLC12A4 | m | ? | Kanaka et al., 2001; Li et al., 2002 |
KCC3 | SLC12A6 | m,p | B | Pearson et al., 2001 | |
KCC4 | SLC12A7 | m,p | A | Karasheh et al., 2002 | |
Cl--HCO3- exchange | AE2 | SLC4A2 | m,p | B | Lindsey et al., 1990; Alper et al., 1994; Wu et al., 1998 |
Na+- HCO3- cotransporters | NBCe2 | SLC4A6 | m | ? | Praetorius et al., 2004 |
NBCn1 | SLC4A7 | m,p | B | Praetorius et al., 2004 | |
NBCE | SLC4A10 | m,p | B | Praetorius et al., 2004 | |
Na+-Hexchange Aquaporins | NHE1 | SLC9 | m | ? | Kalaria et al., 1998 |
AQP1 | AQP1 | m,p | A | Nielsen et al., 1993 | |
AQP4 | AQP4 | m,p | O | Venero et al., 1999; Speake et al., 2003 | |
Kv channels | Kv1.1 | KCNA1 | p | A | Speake et al., 2004 |
Kv1.3 | KCNA3 | p | A | Speake et al., 2004 | |
Kv1.6 | KCNA6 | p | ? | Speake and Brown (unpublished data) | |
Kir channels | Kir7.1 | KCNJ13 | m,p | A | Döring et al., 1998; Nakamura et al., 1999 |
Common names and the HUGO (Human Genome Organisation) names are given. The bold names are transporters for which there is functional evidence in the choroid plexus. mRNA (m) refers to detection by northern analysis, RT-PCR or in situ hybridisation. Protein (p) expression was determined by Western analysis or immunocytochemistry. Mem. is the site of expression within the cell: A, apical membrane; B, basolateral membrane; O, organelle membranes.
Na+-K+ ATPase (ATP1A1, ATP1B1, ATP1B2)
The Na+-K+ ATPase is a heterodimer normally consisting of two polypeptides, one α and one β subunit (Blanco and Mercer, 1998; Scheiner-Bobis, 2002). The α subunit is a multi-spanning membrane protein which contains the ATP and cation binding sites. It is this subunit therefore which mediates the transport of three Na+ out of the cell, and two K+ into the cell at the expense of a single molecule of ATP. The glycoprotein β subunit crosses the plasma membrane only once, but is required for the correct functioning of the pump. The β subunit also modulates the affinity of the pump for K+ and Na+ (Blanco and Mercer, 1998). Four different α subunits (1-4) and three β subunits (1-3) have been identified in mammalian cells (Blanco and Mercer, 1998; Scheiner-Bobis, 2002). A γ subunit, which is also thought to modulate pump activity, has also been observed in some tissues (Scheiner-Bobis, 2002).
In the choroid plexus, activity of the Na+-K+ ATPase pump is closely associated with the secretion of CSF. Inhibitors of the pump, e.g. the cardiac glycoside ouabain, have been shown to reduce CSF secretion, and the movement of Na+ into the CSF (Davson and Segal, 1970;Wright, 1978; Pollay et al., 1985). In RT-PCR and Western blotting studies α1 (ATP1A1), β1 (ATP1B1) and β2 (ATP1B2) subunits have been identified in rat choroid plexus (Watts et al., 1991; Zlokovic et al., 1993; Klarr et al., 1997). The γ subunit has not been observed in choroid plexus; however, phospholemman (a homologue of the γ subunit) does appear to be associated with the Na+-K+ ATPase in choroid plexus (Feschenko et al., 2003).
In immunocytochemical studies the α1 sub-unit was found to be expressed exclusively on the apical brush border membrane in rat and mouse choroid plexus (Masuzawa et al., 1984b; Ernst et al., 1986). This is in marked contrast to most epithelial cells where the Na+-K+ ATPase pump is expressed only in the basolateral membrane (Rizzolo, 1999). The apical localization in the choroid plexus is vital because the pump is the major pathway for Na+ secretion into the CSF. Furthermore, the Na+-K+ ATPase is also the most important mechanism for K+ uptake from the CSF into the epithelial cells. Indeed, the expression of all three of the Na+-K+ ATPase sub-units is increased the choroid plexus tissue isolated from rats with experimentally-induced hyperkalemia (Klarr et al., 1997). This presumably reflects the need to remove more K+ from the CSF in these animals.
Cation-chloride cotransporters (SLC12 family)
These are a family of seven transporters which mediate the electroneutral transport Cl- and cations (Na+ and/or K+)in the same direction across the cell membrane (Hebert et al., 2004). The Na+-Cl- cotransporter (NCC) and Na+-2Cl--K+ cotransporters (NKCC1 and NKCC2) mediate ion influx, driven by the chemical gradient for Na+. The K+-Cl- cotransporters (KCC1-KCC4), on the other hand, mediate ion efflux driven by the chemical gradient for K+. The possible expression of cation-chloride cotransporters in the choroid plexus, and their potential role in CSF secretion has until recently been the subject of some controversy (Javaheri, 1991). It is now known that the choroid plexus epithelium expresses several different cation-chloride cotransporters, in both the apical and basolateral membrane.
Na+-K+-2Cl- cotransporter (SLC12A2)
There is good functional data for the expression of Na+-K+-2Cl- cotransporters in choroid plexus. K+ fluxes in to and out of the choroid plexus are significantly reduced by bumetanide (a specific inhibitor of Na+-K+-2Cl- cotransporters, Bairamian et al., 1991; Keep et al., 1994). Expression of the NKCC1 (SLC12A1) isoform of the Na+-K+-2Cl- cotransporters has been demonstrated in the rat choroid plexus by in situ hybridization and Western analysis (Plotkin et al., 1997). By contrast there is no evidence for the expression of either NKCC2 (SLC12A2) or NCC (SLC12A3) in rat choroid plexus by RT-PCR (Davies and Brown, unpublished observations).
Immunocytochemical studies indicate that the NKCC1 protein is expressed in the apical membrane of epithelial cells from the rat choroid plexus (Plotkin et al., 1997; Wu et al., 1998). This observation, while contrary to some expectations (see Javaheri, 1991), is consistent with the functional data of Keep et al. (1994). The role of the NKCC1 in the apical membrane, however, is unclear. Keep et al. (1994), on the basis of estimates of intracellular ion concentrations, calculated that the cotransporter exports ions from the epithelial cells into the CSF (i.e. contribute to Na+ and Cl- secretion). On the other hand, Wu et al. (1998) found that inhibiting the NKCC1 with bumetanide reduced choroid plexus epithelial cell volume, suggesting that the cotransporter mediates ion transport into the epithelial cell. More recently Angelow et al. (2003) found that Na+-dependent transport of vitamin C into choroid plexus cells was enhanced in the presence of bumetanide. This observation supports the hypothesis that NKCC1 mediates Na+ (K+ and Cl-) influx. The significance of these ion movements in CSF secretion, however, remains to be established.
K+-Cl- cotransporters (SLC12A4, SLC12A6, SLC12A7)
The functional data supporting the possible expression of K+-Cl- cotransporters in mammalian cells is less convincing than that for NKCC1. Zeuthen (1994) found evidence for the cotransport of K+, Cl- and H2O in the apical membrane of amphibian (Necturus maculosus) choroid plexus, but whether this is mediated by an amphibian KCC isoform, remains to be determined. By contrast Keep et al. (1994) was unable to identify DIOA-sensitive components of K+ fluxes into or out of rat choroid plexus epithelial cells (DIOA being a specific KCC inhibitor; Culliford et al., 2003). However, molecular biological methods have demonstrated the expression of KCCs on both the apical and basolateral membranes of the choroid plexus epithelium.
Several in situ hybridization studies have shown that mRNA encoding KCC1 (SLC12A4) is expressed in the choroid plexuses of rats and mice (Kanaka et al., 2001; Li et al., 2002). mRNA has also been identified by RT-PCR (Fig. 3), but KCC1 protein expression has yet to be investigated. The expression of mRNA for KCC4 (SLC12A7) has also been determined in choroid plexus by RT-PCR (Fig. 3) and in situ hybridization (Li et al., 2002). A recent immunocytochemical study has shown that the KCC4 protein is expressed in the apical membrane of mouse choroid plexus (Karadsheh et al., 2004). There is no evidence for the expression KCC2 (SLC12A5) in choroid plexus either by RT-PCR (Fig. 3) or by in situ hybridization (Kanaka et al., 2001).
KCC expression in rat choroid plexus by RT-PCR. The numbers at the top of the figure indicate RT-PCR products obtained with primers specific for (1) KCC1, (2) KCC2, (3a) KCC3a, (3b) KCC3b or (4) KCC4, and mRNA isolated from rat fourth ventricle choroid plexus. The numbers to the left indicate the position of molecular mass markers. All the products were of the expected size, and showed at least 91% identity with respective published sequences for mouse KCCs.
There are two isoforms of KCC3 (SLC12A6): KCC3b is a truncated form of KCC3a (a 50 amino acid deletion at the n-terminal which contains several putative phosphorylation sites; Mount et al., 1999). The functional differences between the two isoforms remain to be determined. Expression of KCC3a, but not KCC3b was determined in mouse choroid plexus by northern analysis (Pearson et al., 2001). By contrast we have found evidence of both KCC3a and KCC3b expression by RT-PCR (see Fig. 3). Pearson et al. (2001) determined that that KCC3 protein is expressed in the basolateral membrane of rat choroid plexus. The antibody used in these experiments did not, however, discriminate between KCC3a and KCC3b, so that both isoforms may be expressed in this membrane.
The potential roles of the KCCs in the apical and basolateral membranes are unclear. A role for KCC1 is difficult to predict without knowledge of the membrane in which the transporter is expressed. The KCC3 transporters in the basolateral membrane may have a significant role in the absorption of K+ from the CSF to the blood, as they are to date the only transporter or channel identified in the basolateral membrane capable of mediating K+ efflux. The role of KCC4 in the apical membrane is less obvious, but they may contribute to Cl- transport into the CSF, and to the recycling of K+ which occurs at this membrane (Zeuthen and Wright, 1981).
HCO3- transporters (SCL4 family)
The transport of HCO3- is known to play a central role in CSF secretion (Brown and Garner, 1993; Saito and Wright, 1983; Saito and Wright, 1984). A diverse range of transport proteins have now been identified which mediate both the influx and efflux of HCO3-; the Cl--HCO3- exchangers, Na+-HCO3- cotransporters and Na+-dependent Cl--HCO3- exchangers. Several of these transporters are now known to be expressed in the choroid plexus. The molecular identity of many of these transporters has only recently been determined, and several isoforms remain incompletely characterized (Romero et al., 2004). Furthermore, an accepted nomenclature of the transporters has yet to be established. In this review we have adopted the nomenclature suggested in the recent review by Romero et al. (2004).
Cl--HCO3- exchanger (SLC4A2)
Cl--HCO3- exchangers are expressed in many different types of cell. Their main function is in the regulation of intracellular pH (i.e. they mediate HCO3- efflux helping to acidify the cytoplasm), but they can also play a role in cell volume regulation and in epithelial transport (Alper et al., 2002). The first indication that Cl--HCO3- exchangers may be involved in CSF secretion came from experiments in which DIDS (an inhibitor of Cl--HCO3- exchange, but also other anion transporters) was found to inhibit Cl- transport into the CSF (Frankel and Kazemi, 1983; Deng and Johanson, 1989). Additional evidence for the expression of Cl--HCO3- exchangers was provided by studies of intracellular pH regulation in rat lateral ventricle choroid plexus epithelial cells (Johanson et al., 1985). As a result of these studies it was suggested that Cl--HCO3- exchangers are expressed in the apical membrane of the choroid plexus, where they would contribute directly to the secretion of HCO3- into the CSF.
Three functional isoforms of the anion exchanger (AE1-AE3) have been isolated: AE1 is expressed in red blood cells, AE2 is the epithelial isoform and AE3 is expressed in neurons. Lindsey et al. (1990) identified the expression of the AE2 isoform in the choroid plexus epithelium. Immunolocalisation showed that the AE2 protein is expressed exclusively in the basolateral membrane of mouse choroid plexus cells (Lindsey et al., 1990), an observation which has since been confirmed by Alper et al. (1994) and Wu et al. (1998) in rat choroid plexus. This observation ended the speculation that the exchanger provides a route for HCO3- entry into the CSF. Lindsey et al. (1990) suggested, instead, that the main role for AE2 in the choroid plexus may be in the regulation of intracellular pH. However, AE2 may also provide an important route for Cl- influx across the basolateral membrane of the choroid plexus. Indirect evidence for this hypothesis was provided by data from single channel, patch clamp studies of rat choroid plexus (Brown and Garner, 1993).
Electrogenic Na+-HCO3- cotransporters (SLC4A4, SLC4A5)
The Na+-HCO3- cotransporters (NBC) are classified as either electrogenic or electroneutral (Romero et al., 2004). An electrogenic transporter is one which generates an electric current, by mediating the net movement of charge across a membrane. In the case of an NBC this means that more then one HCO3- is transported with each Na+.By contrast the electroneutral transporters mediate the movement of an equal number of positive and negative charges.
NBCs were first identified in the kidney proximal tubule (Yoshitomi et al., 1985; Soleimani and Aronson, 1989). The kidney transporter (NBCe1 or SLC4A4) mediates the electrogenic efflux HCO3- from the cell by transporting three HCO3- for one Na+. At this stoichiometry, the outward electrochemical gradient for HCO3- efflux is thermodynamically more favorable than that for the inward movement of Na+. The molecular identity of the kidney NBCe1 was determined by Romero et al. (1997). Since then other NBCe1 isoforms have been identified in pancreas, heart, brain, etc. (Romero et al., 2004). These newer isoforms all exhibit a 2:1 stoichiometry and hence mediate HCO3- influx driven by the Na+ electrochemical gradient. A second family of electrogenic NBC (NBCe2 or SLC4A5) has now also been cloned from cardiac muscle (Pushkin et al., 2000). NBCe2 show a wide tissue distribution (Pushkin et al., 2000), and also display a 2:1 stoichiometry (Sassini et al., 2002; Virkki et al., 2002).
NBCs play a crucial role in the secretion by other HCO3- secreting epithelia, e.g. pancreatic duct cells (Case et al., 2004), but until recently there was little evidence for their involvement in CSF secretion. Mayer and Sanders-Bush (1993) demonstrated that a Na+-dependent, DIDS-sensitive transporter contributes to HCO3- transport into choroid plexus cells during intracellular pH regulation, suggesting that NBCs might be expressed in the choroid plexus. Schmitt et al. (2000) have now used in situ hybridization methods to demonstrate that mRNA for NBCe1 is expressed in rat lateral and fourth ventricle. Speake et al. (2001) also reported preliminary RT-PCR data suggesting NBCe1 expression. More recent RT-PCR experiments, however, have identified mRNA for NBCe2, but not NBCe1 in rat and mouse choroid plexus (Praetorius et al., 2004). At present there are no data on either NBCe1 or NBCe2 protein expression in the choroid plexus. Thus the question as to whether electrogenic NBCs are expressed in choroid plexus remains to be confirmed.
Electroneutral Na+-HCO3- cotransporter (SLC4A7)
More definitive data are available for the expression of the electroneutral NBCn-1 (SLC4A7), which transports one HCO3- with one Na+ (Choi et al., 2000). These transporters were first cloned from human skeletal muscle (Pushkin et al., 1999), and have subsequently been identified in many other tissues (Amlal et al., 1999; Choi et al., 2000). The 1:1 stoichiometry means that they are driven by the inwardly directed Na+ gradient. Praetorius et al. (2004) identified mRNA expression of NBCn-1 in rat and mouse choroid plexus. Furthermore, NBCn-1 protein was found to be expressed in the basolateral membrane of rat and mouse choroid plexus by immunocytochemical methods (Praetorius et al., 2004). The combined actions of NBCn-1 and AE2 in the basolateral membrane, may explain the accumulation of Na+, HCO3- and Cl- into the cytoplasm of the choroid plexus epithelial cell, which is thought to be an important step in CSF secretion (Brown and Garner, 1993; Segal, 1993).
Na dependent Cl--HCO3- exchange (SLC4A10)
The molecular structure of two isoforms of Na dependent Cl--HCO3- exchangers have been determined: NDCBE (SLC4A8; Grichtchenko et al., 2001) and NBCE (SLC4A10; Wang et al., 2000). These transporters mediate the efflux of one Cl- in exchange for the influx of one Na+ and two HCO3-. Praetorius et al. (2004), have recently demonstrated using RT-PCR and Western blotting that NCBE is expressed in rat and mouse choroid plexus. Immunocytochemistry showed that the protein is expressed in the basolateral membrane of the choroid plexus epithelium, where it may act with NBCn1 to help mediate Na+ and HCO3- influx as a first step in the process of CSF secretion.
Na+-H+ exchange (SLC9 family)
The Na+-H+ exchangers are membrane bound electroneutral transport proteins, which under normal physiological conditions, transport H+ out of the cell in exchange for Na+ (Orlowski and Grinstein, 2004). Na+-H+ exchangers are widely expressed and mainly have a housekeeping role within cells, e.g. regulation of intracellular pH and cell volume. However, they also contribute to transepithelial ion transport (Orlowski and Grinstein, 2004). Evidence of Na+-H+ exchanger expression in the choroid plexus comes from the work of Murphy and Johanson (1989,1990), who demonstrated that Na+ transport into the CSF is reduced by amiloride (an inhibitor of the Na+-H+ exchanger). Furthermore, because of the sidedness of the effect of amiloride, it was postulated that the exchanger is expressed in the basolateral membrane of rat lateral and fourth ventricle choroid plexus. Additional evidence for the presence of the exchanger in the rabbit choroid plexus was provided by studies of intracellular pH regulation in epithelial cells maintained in primary culture (Mayer and Sanders-Bush, 1993). The presence of the exchanger in the basolateral membrane would provide a mechanism for Na+ transport into the choroid plexus epithelial cell, in addition to helping to regulate intracellular pH in these cells.
A total of eight isoforms of the Na+-H+ exchanger (NHE-1 to NHE-8) have been identified to date (Orlowski and Grinstein, 2004). The expression of NHE1 in the pig lateral ventricle choroid plexus was indicated by amiloride binding studies and apparently confirmed by RT-PCR methods (Kalaria et al., 1998). Alper et al. (1994), however, found no evidence for the expression of NHE1 in the rat lateral ventricle choroid plexus, using immunocytochemical methods. The molecular identity and membrane localization of the exchanger in the choroid plexus therefore remain unclear.
Carbonic anhydrases
The carbonic anhydrases are a group of enzymes which catalyze the production of HCO3- and H+ from H2O and CO2. Although not directly involved in ion transport, it is thought that carbonic anhydrases have an important role in CSF secretion. The main evidence for this is that acetazolamide, a carbonic anhydrase inhibitor, reduces CSF secretion in rats by as much as 50% (Vogh et al., 1987), and that acetazolamide can also reduce CSF pressure in children with hydrocephalus (Cowan and Whitelaw, 1991).
There are at least seven mammalian carbonic anhydrase isozymes with different tissue distributions and intracellular locations. Histochemical methods were first used to demonstrate carbonic anhydrase expression in the cytoplasm and membrane of the rat choroid plexus (Masuzawa and Sato, 1983). Immunocytochemical methods have subsequently identified CAII in the cytoplasm of rat choroid plexus epithelial cells (Masuzawa et al., 1984a), and CAIII in rat lateral ventricle and human choroid plexus (Nogradi et al., 1993) in the cytoplasm of the epithelial cells. The expression of the acetazolamide-insensitive CAIII isozyme may explain why acetazolamide only partially inhibits CSF secretion (Nogradi et al., 1993). Functional studies suggest that the membrane bound isozyme CAIV contributes little to CSF secretion in the rat (Maren et al., 1997).
Aquaporins
The expression of the aquaporins has not been systematically studied in choroid plexus. However, there is evidence that AQP1 and AQP4 are expressed in this epithelium
The expression of AQP1 has been determined in the apical membrane of the rat choroid plexus epithelium using immunocytochemical methods (Nielsen et al., 1993; Wu et al., 1998; Speake et al., 2003). Furthermore, Mobasheri and Marples (2004) have demonstrated that the choroid plexus exhibits the highest expression of AQP1 of any human tissue using microarray methods. AQP1 is therefore likely to have a major role in mediating water transport across the apical membrane during CSF secretion. Indeed, it has recently been shown that CSF production is significantly reduced in transgenic mice in which AQP1 has been deleted (Oshio et al., 2003).
AQP4 is widely expressed in the brain (Badaut et al., 2002). It is also expressed in the basolateral membrane of many epithelial cells (Frigeri et al., 1995). It is therefore a prime candidate as the route for water transport across the basolateral membrane of the choroid plexus. Data on AQP4 expression in the choroid plexus, however, are equivocal. In situ hybridization studies have provided evidence for (Venero et al., 1999) and against (Hasegawa et al., 1994). Similarly, studies of protein expression are unclear. Frigeri et al. (1995) and Nielsen et al. (1997) reported no expression in choroid plexus by immunocytochemistry, although it was found to be expressed in basolateral membrane of ependymal cells which line the ventricles of the brain (Nielsen et al., 1997). By contrast, Speake et al. (2003) found evidence of AQP4 protein expression in choroid plexus by Western analysis. Immunocytochemical methods also demonstrated some expression of AQP4 in rat choroid plexus cells. Expression, however, was confined to the cytoplasm (possibly in organellar membranes), and AQP4 did not appear to be expressed in either the apical or basolateral membrane (Speake at al, 2003). The route for water transport across the basolateral membrane of the choroid plexus therefore remains to be determined.
K+ channels
K+ channels are thought to have a number of important roles in CSF secretion by the choroid plexuses. First, they help generate the negative membrane potential (Vm), and hence contribute to the electrochemical gradient favoring anion efflux at the apical membrane. Second, they act as leak pathway for K+ accumulated in the cell through the actions of the Na+-K+ ATPase. Finally, K+ channels may participate in the transcellular transport (CSF to blood) of K+, a process which is vital in maintaining the low [K+]of the CSF (Zeuthen and Wright, 1981). Patch clamp experiments have identified two major components to the whole-cell K+ conductance in rat choroid plexus. Fig. 4A shows that a time independent, inward-rectifying conductance (Kir) is observed at hyperpolarizing Vm, while at depolarising Vm a time-dependent outward-rectifying conductance (Kv) is observed. Significant progress has been made over the last 5 years in determining which channel proteins carry these conductances.
Whole-cell conductances in rat choroid plexus epithelial cells. (A) Current profiles for the two components of the K+ conductance (Kv and Kir). The profiles were produced by applying voltage steps from -120 to 60 mV and using a K+-rich electrode solution and an artificial CSF in the bath. Currents via the time-dependent, outward-rectifying conductance (Kv) are observed at potentials greater than -20 mV. The time-independent, inward-rectifying currents (Kir) are observed at potentials of less than -80 mV. (B) The inward-rectifying anion conductance. Currents were recorded in K+-free solutions at Vm= 0 to -140 mV. The dashed line indicates 0 current in and (A) and (B).
Kir 7.1 channels (KCNJ13)
The time-independent, inward-rectifying conductance in choroid plexus shares many properties with Kir channels (Kotera and Brown, 1994). Kir channels are expressed in many mammalian cells and are mainly involved in maintaining Vm. The Kir channel family is divided into seven subfamilies (Kir1-Kir7). All Kir channels have which have a similar molecular structure, i.e. each Kir protein characteristically has two transmembrane spanning domains, with four proteins assembling to produce a functional channel (Coetzee et al., 1999).
The first indication that the Kir conductance in choroid plexus was carried by the Kir7.1 came from in situ hybridization studies which showed that Kir7.1 is highly expressed in the choroid plexus epithelium (Döring et al., 1998). In functional studies, Döring et al. (1998) showed that Kir7.1, when expressed in Xenopus oocytes, gives rise to an inward-rectifying conductance with properties that are almost identical to those of the Kir in the choroid plexus. This strongly suggests that Kir7.1 makes a major contribution to the Kir conductance in the choroid plexus. Nakamura et al. (1999) demonstrated that the Kir7.1 channels are expressed in the apical membrane of the choroid plexus using immunocytochemical methods. These channels must therefore contribute to K+ efflux at this membrane.
Kv1 family of channels
Kv channels are expressed in many excitable and nonexcitable cells. They are members of the six transmembrane-spanning domain family of voltage-gated and Ca2+-activated K+ channels (Coetzee et al., 1999). All Kv channels are activated by depolarisation and exhibit outward-rectification. In the choroid plexus, Kv channels contribute to the time-dependent, outward-rectifying K+ conductance (Fig. 4A). This conductance is activated by depolarising Vm, but is Ca2+-independent (Kotera and Brown, 1994).
The properties of the Kv conductance in choroid plexus are similar to those of the Kv1 family of channels (Coetzee et al., 1999). Western analysis was therefore used to investigate Kv1 protein expression. Kv1.1 (KCNA1), Kv1.3 (KCNA3) and Kv1.6 (KCNA6) were all identified by this method in rat choroid plexus (Fig. 5), but not Kv1.4 and Kv1.5 (Kv1.2 expression has not been determined). Furthermore, the whole-cell K+ currents in choroid plexus were partially inhibited by both dendrotoxin-K and margatoxin (highly specific blockers of Kv1.1 and Kv1.3 channels respectively; Speake et al., 2004). These observations suggest that Kv1.1, Kv1.3 and possibly Kv1.6 may contribute to the whole cell K+ conductance. Immunocytochemical studies have demonstrated that the expression of Kv1.1 and Kv1.3 is confined to the apical membrane of rat choroid plexus (Speake et al., 2004). Thus, Kv1.1 and Kv1.3 channels are thought to contribute to K+ efflux at the apical membrane of the choroid plexus.
Kv1.1, Kv1.3 and Kv1.6 are expressed in the choroid plexus epithelium. Western analysis was performed a membrane rich fraction isolated from rat fourth ventricle choroid plexus using specific antibodies for Kv1.1, Kv1.3 and Kv1.6. +Ag refers to Western analyses performed when the primary antibody was pre-adsorbed with an excess of the antigens to which each antibody was raised.
Anion channels
Anion channels are a vital component of the secretory process in most epithelia (Jentsch et al., 2002). This is vividly demonstrated in the disease cystic fibrosis, where a defect in the anion channel expression in apical membranes of certain epithelia results in a profound reduction in fluid secretion. The anion channel affected in cystic fibrosis is CFTR (cystic fibrosis transmembrane conductance regulator), which is activated by cAMP (Jentsch et al., 2002). In other epithelia Ca2+ activated Cl- channels play crucial roles in secretion (Jentsch et al., 2002). It is therefore probable that anion channels are involved in CSF secretion; however, neither CFTR nor Ca2+-activated Cl- channels are expressed in the choroid plexus (Kibble et al., 1996, 1997). Two other types of anion channel are expressed: i) an inward-rectifying anion channel, and ii) a volume-sensitive anion channel. At least one of these channels is thought to have a significant role in CSF secretion.
Inward-rectifying anion channels
Anion channels with inward-rectifying current-voltage relationships have been observed in whole cell recordings from the choroid plexus of rat (Kibble et al., 1996), mouse (Kibble et al., 1997), pig (Kajita et al., 2000a) and the amphibian N. maculosus (Birnir et al., 1989). These channels: i) exhibit time-dependent activation at hyperpolarizing Vm (Fig. 4B), ii) have a uniquely high permeability to HCO3- (PHCO3:PCl=1.5), iii) are more permeant to I- than Cl- or Br-; iv) are blocked by: Cl- channel blockers (DIDS or the anthracene derivative NPPB), divalent cations (Ba2+, Cd2+ and Zn2+) and H+; v) are activated by cAMP and protein kinase A, but inhibited by protein kinase C (Kibble et al., 1996; Kajita and Brown, 1997; Kajita et al., 2000b). Many of these properties are similar to those of the ClC-2 channel (Jentsch et al., 2002), mRNA for which is expressed the choroid plexus (Smith et al., 1995; Speake et al., 2002). However, the inward-rectifying conductance was unchanged in whole cell recordings in choroid plexus cells from ClC-2 knock-out mice, indicating that ClC2 channels do not contribute to the conductance (Speake et al., 2002). Thus, the molecular identity of the channel remains unknown. The properties of the channel are such that it may have a unique molecular structure.
The inward-rectifying channels make a significant contribution to the whole-cell conductance at the resting Vm (Kibble et al., 1996), suggesting that they have a role in CSF secretion. Furthermore, the regulation of these channels by cAMP and the high permeability to HCO3-, suggest that they are similar to the HCO3- channel which has a major role in CSF secretion by bullfrog choroid plexus (Saito and Wright, 1984). The properties are also consistent with the observation that Cl- efflux from the rat choroid plexus is stimulated by cAMP (Deng and Johanson, 1992), and inhibited by agonists such as vasopressin which activate protein kinase C (Johanson et al., 1999). To participate in CSF secretion the inward-rectifying channels must be located in the apical membrane; however, the site of their expression has yet to be determined.
Volume-sensitive anion channels
Volume-sensitive anion channels are also expressed in choroid plexus cells from rats and mice (Kibble et al., 1996,1997). These channels are activated by cell swelling, and activity is dependent on intracellular ATP (Kibble et al., 1997). The channels are blocked by DIDS and NPPB (Kibble et al., 1997). These properties are very similar to those of volume-sensitive anion channels found in many cells (Okada, 1997; Jentsch et al., 2002). Volume-sensitive channels have an important role in the regulatory volume decrease observed in most cells in response to cell swelling (Okada, 1997; Jentsch et al., 2002). The potential roles of the volume-sensitive anion channels in choroid plexus epithelial cells, however, have not been investigated.
A model of CSF secretion
Fig. 6 summarizes the data on ion transport protein expression in the choroid plexus epithelium, and provides working models of how these transporters interact to bring about CSF secretion and K+ absorption. The major ion fluxes across the choroid plexus epithelium are detailed in Fig. 6A, while Fig. 6B provides a model for the unidirectional transport of Na+, HCO3- and Cl- from the blood to CSF side of the epithelium. Fig. 6B is based on the model first proposed by Saito and Wright (1983) for CSF secretion by the bullfrog choroid plexus. The model has gradually evolved over the last 20 years, and includes nearly all of what has subsequently been determined about ion transporter and channel expression in the mammalian choroid plexus. The Na+-K+ ATPases in the apical membrane are central to the model in Fig. 6B. These Na+ pumps are the only route for Na+ transport into the CSF; they also generate the energy, in the form of the electrochemical gradient for Na+, which either directly or indirectly drive the events at the basolateral membrane of the epithelium.
Ion transport by the choroid plexus. (A) Major fluxes of ions across the choroid plexus epithelium. (B) Ion transporters involved in Na+, HCO3- and Cl- secretion by the choroid plexus. c.a., carbonic anhydrase. (C) Mechanism of K+ absorption. (D) H2O transport in the choroid plexus epithelium.
Events at the basolateral membrane.
A wide range of transporters are expressed in the basolateral membrane of the choroid plexus epithelium (Fig. 6B). The net action of these proteins is to mediate the transport of Na+, HCO3- and Cl- into the epithelial cells. The NBCn1 and NBCE use the Na+ gradient to drive the accumulation of HCO3-. HCO3- is also produced within the epithelial cells through the actions of carbonic anhydrase (H+ produced as a by-product are removed from the cell via the Na+-H+ exchange in the basolateral membrane). The resultant outwardly directed, HCO3- gradient drives Cl- transport into the cell on the AE2 in the basolateral membrane. Thus, the overall action of the transporters is to mediate the Na+- and HCO3--dependent accumulation of Cl-.
Events at the apical membrane.
The Na+-K+ AT-Pase pumps in the apical membrane are responsible for the transport of Na+ out of the cell into the CSF. HCO3- and Cl- leave the cell down their respective electrochemical gradients via the inward-rectifying anion channels in the apical membrane of the epithelium. Cl- efflux may also take place via KCC4 which is expressed in the apical membrane. H2O is transported across the apical membrane of the epithelium via AQP1. The route for H2O transport across the basolateral membrane, however, remains to be determined (Fig. 6D). One possibility is that water moves via the solute carriers expressed in the basolateral membrane of the choroid plexus (see MacAulay et al., 2004).
Model of K+ absorption from the CSF by the choroid plexus
The absorption of K+ across the choroid plexus epithelium can also be explained by our knowledge of transporter and channel expression (Fig. 6C). The activity of the Na+-K+ ATPasea is once again central to the model in Fig. 6C. They are the main mechanism by which K+ can be transported, against a large electrochemical gradient, from the CSF into the epithelial cells. NKCC1 in the apical membrane may also contribute to K+ uptake. Once inside the epithelial cell, the vast majority of this K+ is recycled across the apical membrane. Zeuthen and Wright (1981) suggested that more than 90% of the K+ leaves bullfrog epithelial cells via K+ channels in the apical membrane. In the mammalian choroid plexus the Kv1 channels and Kir7.1 probably provide the major route for K+ efflux. However, KCC4 may also contribute to K+ efflux at the apical membrane. This recycling of K+ is necessary to limit the transport of K+ across the epithelium, so that the CSF does not become denuded of K+.
To explain K+ absorption, however, some of the K+ pumped into the cell via the Na+-K+ ATPases must leave across the basolateral membrane (Fig. 6C). Zeuthen and Wright (1981) reported that ion channels in the basolateral membrane (i.e. approximately 10% of the total K+ conductance) were responsible for K+ absorption in bullfrog choroid plexus. However, K+ channels have not been identified in the basolateral membrane of the mammalian choroid plexus. Thus, the KCC3 may be the main route for K+ efflux at the basolateral membrane in mammals (Fig. 6C). KCC1 may also contribute to K+ efflux if it is expressed in the basolateral membrane.
CONCLUSIONS
This review has shown how our knowledge of ion transport protein expression in the choroid plexus epithelium has increased over the last 15 years. The ways in which these newly identified proteins can be incorporated into models of ion transport (Fig. 6B, C), have also been discussed. The fact that these models are simply refinements of older models is reassuring, and suggests that we may be getting close to a comprehensive understanding of ion transport by the choroid plexus. However, it is important to remember that the models in Fig. 6 have not to been subjected to rigorous experimental testing, and this is the next challenge facing physiologists studying ion transport by the choroid plexus. This challenge will certainly be helped by the newfound abilities to maintain isolated choroid plexus cells in culture as epithelial monolayers (Haselbach et al., 2001), i.e. these preparations will greatly simplify measurements of ion fluxes across the epithelium. A second challenge facing cell physiologists is to understand how ion concentrations in the CSF are regulated by the choroid plexuses. The use of epithelial monolayers once again may be vital in determining whether the concentrations of K+ and HCO3- are regulated because of the innate properties of the transporters in the choroid plexus, or whether external factors e.g. nerves and paracrine transmitters, are involved. In conclusion, molecular methods have been useful in identifying transport proteins in the choroid plexus epithelium, but the precise role of many of these proteins in the CSF secretion remains to be elucidated.
Acknowledgments
Acknowledgments-This work was supported by grant 070139/Z/02 from the Wellcome Trust. Sarah Davies is the recipient of an MRC postgraduate scholarship.
Footnotes
Abbreviations:
- CFTR
- cystic fibrosis transmembrane conductance regulator
- CSF
- cerebrospinal fluid.
REFERENCES
- Amlal H, Burnham CE, Soleimani M. Characterization of Na+/HCO-3 cotransporter isoform NBC-3. Am J Physiol. 1999;276:F903–F913. [PubMed] [Google Scholar]
- Alper SL, Stuart-Tilley A, Simmons CF, Brown D, Drenckhahn D. The fodrin-ankyrin cytoskeleton of choroid plexus preferentially colocalizes with apical Na+K+-ATPase rather than with basolateral anion exchanger AE2. J Clin Invest. 1994;93:1430–1438. [PMC free article] [PubMed] [Google Scholar]
- Alper SL, Darman RB, Chernova MN, Dahl NK. The AE gene family of Cl-/HCO3- exchangers. J Nephrol. 2002;15:S41–S53. [PubMed] [Google Scholar]
- Angelow S, Haselbach M, Galla HJ. Functional characterisation of the active ascorbic acid transport into cerebrospinal fluid using primary cultured choroid plexus cells. Brain Res. 2003;988:105–113. [PubMed] [Google Scholar]
- Bairamian D, Johanson CE, Parmalee JT, Epstein MH. Potassium cotransport with sodium and chloride in the choroid plexus. J Neurochem. 1991;56:1632–1629. [PubMed] [Google Scholar]
- Badaut J, Lasbennes F, Magistretti PJ, Regli L. Aquaporins in brain: distribution, physiology and pathophysiology. J Cereb Blood Flow Metab. 2002;22:367–378. [PubMed] [Google Scholar]
- Birnir B, Loo DDF, Brown PD, Wright EM. Whole cell currents in Necturus choroid plexus. FASEB J. 1989;2:A1722. [Google Scholar]
- Blanco G, Mercer RW. Isozymes of the Na-K-ATPase: heterogeneity in structure, diversity in function. Am J Physiol. 1998;275:F633–F650. [PubMed] [Google Scholar]
- Brown PD, Garner C. Cerebrospinal fluid secretion: the transport of fluid and electrolytes by the choroid plexus. In: Oksche A, Rodriguez EM, Fernandez-Llebrez P, editors. The subcommissural organ. 1993. pp. 233–242. Heidelberg: Springer International. [Google Scholar]
- Case RM, Ishiguro H, Steward MC. Pancreatic bicarbonate secretion. In: Johnson LR, editor. Encyclopedia of gastroenterology. Academic Press; New York: 2004. pp. 38–40. [Google Scholar]
- Choi I, Aalkjaer C, Boulpaep EL, Boron WF. An electroneutral sodium/bicarbonate cotransporter NBCn1 and associated sodium channel. Nature. 2000;405:571–575. [PubMed] [Google Scholar]
- Coetzee WA, Amarillo Y, Chiu J, Chow A, Lau D, McCormack T, Moreno H, Nadal MS, Ozaita A, Pountney D, Saganich M, VegaSaenz De Miera E, Rudy B. Molecular diversity of K+ channels. Ann NY Acad Sci. 1999;868:233–285. [PubMed] [Google Scholar]
- Cowan F, Whitelaw A. Acute effects of acetazolamide on cerebral blood flow velocity and pCO2 in the newborn infant. Acta Pediatr Scand. 1991;80:22–27. [PubMed] [Google Scholar]
- Culliford S, Ellory C, Lang HJ, Englert H, Staines H, Wilkins R. Specificity of classical and putative Cl- transport inhibitors on membrane transport pathways in human erythrocytes. Cell Physiol Biochem. 2003;13:181–188. [PubMed] [Google Scholar]
- Davson H, Segal MB. The effects of some inhibitors and accelerators of sodium transport on the turnover of 22Na in the cerebrospinal fluid and the brain. J Physiol. 1970;209:139–153. [PMC free article] [PubMed] [Google Scholar]
- Davson H, Welch K, Segal MB. The physiology and pathophysiology of the cerebrospinal fluid. Churchill Livingston; Edinburgh: 1987. [Google Scholar]
- Deng QS, Johanson CE. Stilbenes inhibit exchange of chloride between blood, choroid plexus and the cerebrospinal fluid. Brain Res. 1989;510:183–187. [PubMed] [Google Scholar]
- Deng QS, Johanson CE. Cyclic AMP alteration of chloride transport into the choroid plexus-cerebrospinal fluid system. Neurosci Lett. 1992;143:146–150. [PubMed] [Google Scholar]
- Döring F, Derst C, Wischmeyer E, Karschin C, Schneggenburger R, Daut J, Karschin A. The epithelial inward rectifier channel Kir 7.1 displays unusual K+ permeation properties. J Neurosci. 1998;18:8625–8636. [PMC free article] [PubMed] [Google Scholar]
- Ernst SA, Palacios JR, Siegel GJ. Immunocytochemical localization of Na+, K+-ATPase catalytic polypeptide in mouse choroid plexus. J Histochem Cytochem. 1986;34:189–195. [PubMed] [Google Scholar]
- Frankel H, Kazemi H. Regulation of CSF composition-blocking chloride-bicarbonate exchange. J Appl Physiol. 1983;55:177–182. [PubMed] [Google Scholar]
- Feschenko MS, Donnet C, Wetzel RK, Asinovski NK, Jones LR, Sweadner KJ. Phospholemman, a single-span membrane protein, is an accessory protein of Na, K-ATPase in cerebellum and choroid plexus. J Neurosci. 2003;23:2161–2169. [PMC free article] [PubMed] [Google Scholar]
- Frigeri A, Gropper MA, Umenishi F, Kawashima M, Brown D, Verkman AS. Localization of MIWC and GLIP water channel homologs in neuromuscular, epithelial and glandular tissues. J Cell Sci. 1995;108:2993–3002. [PubMed] [Google Scholar]
- Grichtchenko II, Choi I, Zhong X, Bray-Ward P, Russell JM, Boron WF. Cloning, characterization, and chromosomal mapping of a human electroneutral Na+-driven Cl-HCO3 exchanger. J Biol Chem. 2001;276:8358–8363. [PubMed] [Google Scholar]
- Hebert SC, Mount DB, Gamba G. Molecular physiology of cation-coupled Cl- cotransport: the SLC12 family. Pflügers Arch. 2004;447:580–593. [PubMed] [Google Scholar]
- Haselbach M, Wegener J, Decker S, Engelbertz C, Galla HJ. Porcine Choroid plexus epithelial cells in culture: regulation of barrier properties and transport processes. Microsc Res Tech. 2001;52:137–152. [PubMed] [Google Scholar]
- Hasegawa H, Ma T, Skach W, Matthay MA, Verkman AS. Molecular cloning of a mercurial-insensitive water channel expressed in selected water-transporting tissues. J Biol Chem. 1994;269:5497–5500. [PubMed] [Google Scholar]
- Husted RF, Reed DJ. Regulation of cerebrospinal fluid potassium by the cat choroid plexus. J Physiol. 1976;259:213–221. [PMC free article] [PubMed] [Google Scholar]
- Husted RF, Reed DJ. Regulation of cerebrospinal fluid bicarbonate by the cat choroid plexus. J Physiol. 1977;267:411–428. [PMC free article] [PubMed] [Google Scholar]
- Javaheri S. Role of NaCl cotransport in cerebrospinal fluid production: effects of loop diuretics. J Appl Physiol. 1991;71:795–800. [PubMed] [Google Scholar]
- Jentsch TJ, Stein V, Weinreich F, Zdebik AA. Molecular structure and physiological function of chloride channels. Physiol Rev. 2002;82:503–568. [PubMed] [Google Scholar]
- Johanson CE. Choroid plexus. In: Adelman G, Smith BH, editors. Encyclopedia of neuroscience. Elsevier Science; New York: 1999. pp. 384–387. [Google Scholar]
- Johanson CE, Parandoosh Z, Smith QR. Cl-HCO3 exchange in choroid plexus: analysis by the DMO method for cell pH. Am J Physiol. 1985;249:F478–F484. [PubMed] [Google Scholar]
- Johanson CE, Preston JE, Chodobski A, Stopa EG, SzmydyngerChodobski J, McMillan PN. AVP V1 receptor-mediated decrease in Cl- efflux and increase in dark cell number in choroid plexus epithelium. Am J Physiol. 1999;276:C82–C90. [PubMed] [Google Scholar]
- Jones HC, Keep F. The control of potassium concentration in the cerebrospinal fluid and brain interstitial fluid of developing rats. J Physiol. 1987;383:441–453. [PMC free article] [PubMed] [Google Scholar]
- Kajita H, Brown PD. Inhibition of the inward-rectifying Cl- channel in the rat choroid plexus by a decrease in extracellular pH. J Physiol. 1997;498:703–707. [PMC free article] [PubMed] [Google Scholar]
- Kajita H, Omori K, Matsuda H. The chloride channel ClC-2 contributes to the inwardly rectifying Cl- conductance in cultured porcine choroid plexus epithelial cells. J Physiol. 2000a;523:313–324. [PMC free article] [PubMed] [Google Scholar]
- Kajita H, Whitwell C, Brown PD. Properties of the inward-rectifying Cl- channel in rat choroid plexus: regulation by intracellular messengers and inhibition by divalent cations. Pflügers Arch. 2000b;440:933–940. [PubMed] [Google Scholar]
- Kalaria RN, Premkumar DR, Lin CW, Kroon SN, Bae JY, Sayre LM, LaManna JC. Identification and expression of the Na+/H+ exchanger in mammalian cerebrovascular and choroidal tissues: characterisation by amiloride-sensitive [3H]MIA binding and RT-PCR analysis. Brain Res Mol Brain Res. 1998;58:178–187. [PubMed] [Google Scholar]
- Kanaka C, Ohno K, Okabe A, Kuriyama K, Itoh T, Fukuda A, Sato K. The differential expression patterns of messenger RNAs encoding K-Cl cotransporters (KCC1,2) and Na-K-2Cl cotransporter (NKCC1) in the rat nervous system. Neuroscience. 2001;104:933–946. [PubMed] [Google Scholar]
- Karadsheh MF, Byun N, Mount DB, Delpire E. Localization of the KCC4 potassium-chloride cotransporter in the nervous system. Neuroscience. 2004;123:381–391. [PubMed] [Google Scholar]
- Keep RF, Xiang J, Betz AL. Potassium cotransport at the rat choroid plexus. Am J Physiol. 1994;267:C1616–C1622. [PubMed] [Google Scholar]
- Kibble JD, Tresize AO, Brown PD. Properties of the cAMP-activated Cl- conductance in choroid plexus epithelial cells isolated from the rat. J Physiol. 1996;496:69–80. [PMC free article] [PubMed] [Google Scholar]
- Kibble JD, Garner C, Kajita H, Colledge WH, Evans MJ, Radcliff R, Brown PD. Whole-cell Cl- conductances in mouse choroid plexus epithelial cells do not require CFTR expression. Am J Physiol. 1997;272:C1899–C1907. [PubMed] [Google Scholar]
- Klarr SA, Ulanski LJ, Stummer W, Xiang J, Betz AL, Keep RF. The effects of hypo- and hyperkalemia on choroid plexus potassium transport. Brain Res. 1997;758:39–44. [PubMed] [Google Scholar]
- Kotera T, Brown PD. Two types of potassium current in rat choroid plexus epithelial cells. Pflügers Arch. 1994;237:317–324. [PubMed] [Google Scholar]
- Li H, Tornberg J, Kaila K, Airaksinen MS, Rivera C. Patterns of cation-chloride cotransporter expression during embryonic rodent CNS development. Eur J Neurosci. 2002;16:2358–2370. [PubMed] [Google Scholar]
- Lindsey AE, Schneider K, Simmons DM, Baron R, Lee BS, Kopito RR. Functional expression and subcellular localization of an anion exchanger cloned from choroid plexus. Proc Natl Acad Sci USA. 1990;87:5278–5282. [PMC free article] [PubMed] [Google Scholar]
- MacAulay N, Hamann S, Zeuthen T. Water transport in the brain: role of cotransporters. Neuroscience. 2004;129:1031–1044. [PubMed] [Google Scholar]
- Maren TH, Conroy CW, Wynns GC, Godman DR. Renal and cerebrospinal fluid formation pharmacology of a high molecular weight carbonic anhydrase inhibitor. J Pharmacol Exp Ther. 1997;280:98–104. [PubMed] [Google Scholar]
- Masuzawa T, Hasegawa T, Nakahara N, Iida K, Sato F. Localization of carbonic anhydrase in rat choroid plexus epithelial cells. Ann NY Acad Sci. 1984a;429:405–407. [PubMed] [Google Scholar]
- Masuzawa T, Ohta T, Kawamura M, Nakahara N, Sato F. Immunohistochemical localization of Na+, K+-ATPase in the choroid plexus. Brain Res. 1984b;302:357–362. [PubMed] [Google Scholar]
- Masuzawa T, Sato F. The enzyme histochemistry of the choroid plexus. Brain. 1983;106:55–99. [PubMed] [Google Scholar]
- Mayer SE, Sanders-Bush E. Sodium-dependent antiporters in choroid plexus epithelial cultures from rabbit. J Neurochem. 1993;60:1308–1316. [PubMed] [Google Scholar]
- Mobasheri A, Marples D. Expression of the AQP-1 water channel in normal human tissues: a semi-quantitative study using tissue mircroarray technology. Am J Physiol. 2004;286:C529–C537. [PubMed] [Google Scholar]
- Mount DB, Mercado A, Song L, Xu J, George AL, Jr, Delpire E, Gamba G. Cloning and characterization of KCC3 and KCC4, new members of the cation-chloride cotransporter gene family. J Biol Chem. 1999;274:16355–16362. [PubMed] [Google Scholar]
- Murphy VA, Smith QR, Rapoport SI. Homeostasis of brain and cerebrospinal fluid calcium concentrations during chronic hypo- and hypercalcemia. J Neurochem. 1986;47:1735–1741. [PubMed] [Google Scholar]
- Murphy VA, Johanson CE. Alteration of sodium transport by the choroid plexus with amiloride. Biochim Biophys Acta. 1989;979:187–192. [PubMed] [Google Scholar]
- Murphy VA, Johanson CE. Na+-H+ exchange in choroid plexus and CSF in acute metabolic acidosis or alkalosis. Am J Physiol. 1990;258:F1528–F1537. [PubMed] [Google Scholar]
- Nakamura N, Suzuki Y, Sakuta H, Ookata K, Kawahara K, Hirose S. Inwardly rectifying K+ channel Kir7.1 is highly expressed in thyroid follicular cells, intestinal epithelial cells and choroid plexus epithelial cells: implication for a functional coupling with Na+, K+-ATPase. Biochem J. 1999;342:329–336. [PMC free article] [PubMed] [Google Scholar]
- Nielsen S, Smith BL, Christensen EI, Agre P. Distribution of the aquaporin CHIP in secretory and resorptive epithelia and capillary endothelia. Proc Natl Acad Sci USA. 1993;90:7275–7279. [PMC free article] [PubMed] [Google Scholar]
- Nielsen S, Nagelhus EA, Amiry-Moghaddam M, Bourque C, Agre P, Ottersen OP. Specialized membrane domains for water transport in glial cells: high-resolution immunogold cytochemistry of aquaporin-4 in rat brain. J Neurosci. 1997;17:171–180. [PMC free article] [PubMed] [Google Scholar]
- Nogradi A, Kelly C, Carter ND. Localization of acetazolamideresistant carbonic anhydrase III in human and rat choroid plexus by immunocytochemistry and in situ hybridisation. Neurosci Lett. 1993;151:162–165. [PubMed] [Google Scholar]
- Okada Y. Volume expansion-sensing outward-rectifier Cl- channel: fresh start to the molecular identity and volume sensor. Am J Physiol. 1997;273:C755–C789. [PubMed] [Google Scholar]
- Orlowski J, Grinstein S. Diversity of the mammalian sodium/proton exchanger SLC9 gene family. Pflügers Arch. 2004;447:549–565. [PubMed] [Google Scholar]
- Oshio K, Song Y, Verkman AS, Manley GT. Aquaporin-1 deletion reduces osmotic water permeability and cerebrospinal fluid production. Acta Neurchir Suppl. 2003;86:525–528. [PubMed] [Google Scholar]
- Pearson MM, Lu J, Mount DB, Delpire E. Localization of the K+-Cl- cotransporter, KCC3, in the central and peripheral nervous systems: expression in the choroid plexus, large neurons and white matter tracts. Neuroscience. 2001;103:481–491. [PubMed] [Google Scholar]
- Plotkin MD, Kaplan MR, Peterson LN, Gullans SR, Hebert SC, Delpire E. Expression of the Na+-K+-2Cl- cotransporter BSC2 in the nervous system. Am J Physiol. 1997;272:C173–C183. [PubMed] [Google Scholar]
- Pollay M, Hisey B, Reynolds E, Tomkins P, Stevens A, Smith R. Choroid plexus Na+/K+-activated adenosine triphosphatase and cerebrospinal fluid secretion. Neurosurgery. 1985;17:768–772. [PubMed] [Google Scholar]
- Praetorius J, Nejsum LN, Nielsen S. A SCL4A10 gene product maps selectively to the basolateral membrane of choroid plexus epithelial cells. Am J Physiol. 2004;286:C601–C610. [PubMed] [Google Scholar]
- Pushkin A, Abuladze N, Lee I, Newman D, Hwang J, Kurtz I. Cloning, tissue distribution, genomic organization, and functional characterization of NBC3, a new member of the sodium bicarbonate cotransporter family. J Biol Chem. 1999;274:16569–16575. [PubMed] [Google Scholar]
- Pushkin A, Abuladze N, Newman D, Lee I, Xu G, Kurtz I. Cloning, characterization and chromosomal assignment of NBC4, a new member of the sodium bicarbonate cotransporter family. Biochim Biophys Acta. 2000;1493:215–218. [PubMed] [Google Scholar]
- Rizzolo LJ. Polarization of the Na+, K+-ATPase in epithelia derived from the neuroepithelium. Int Rev Cytol. 1999;185:195–235. [PubMed] [Google Scholar]
- Romero MF, Hediger MA, Boulpaep EL, Boron WF. Expression cloning and characterization of a renal electrogenic Na+/HCO3 cotransporter. Nature. 1997;387:409–413. [PubMed] [Google Scholar]
- Romero MF, Fulton CM, Boron WF. The SLC4 family of HCO3- transporters. Pflügers Arch. 2004;447:495–509. [PubMed] [Google Scholar]
- Saito Y, Wright EM. Bicarbonate transport across the frog choroid plexus and its control by cyclic nucleotides. J Physiol. 1983;336:635–648. [PMC free article] [PubMed] [Google Scholar]
- Saito Y, Wright EM. Regulation of bicarbonate transport across the brush border membrane of the bull-frog choroid plexus. J Physiol. 1984;350:327–342. [PMC free article] [PubMed] [Google Scholar]
- Sassani P, Pushkin A, Gross E, Gomer A, Abuladze N, Dukkipati R, Carpenito G. Functional characterization of NBC4: a new electrogenic sodium-bicarbonate cotransporter. Am J Physiol. 2002;282:C408–C416. [PubMed] [Google Scholar]
- Scheiner-Bobis G. The sodium pump: its molecular properties and mechanics of ion transport. Eur J Biochem. 2002;269:2424–2433. [PubMed] [Google Scholar]
- Schmitt BM, Berger UV, Douglas RM, Bevensee MO, Hediger MA, Haddad GG, Boron WF. Na/HCO3 cotransporters in rat brain: expression in glia, neurons, and choroid plexus. J Neurosci. 2000;20:6839–6848. [PMC free article] [PubMed] [Google Scholar]
- Segal MB. Extracellular and cerebrospinal fluid. J Inherit Metabol Dis. 1993;16:617–638. [PubMed] [Google Scholar]
- Smith RL, Clayton GH, Wilcox CL, Escudero KW, Staley KJ. Differential expression of an inwardly rectifying chloride conductance in rat brain neurons: a potential mechanism for cell-specific modulation of postsynaptic inhibition. J Neurosci. 1995;15:4057–4067. [PMC free article] [PubMed] [Google Scholar]
- Soleimani M, Aronson PS. Ionic mechanism of Na+-HCO3- cotransport in rabbit renal basolateral membrane vesicles. J Biol Chem. 1989;264:18302–18308. [PubMed] [Google Scholar]
- Speake T, Freeman LJ, Brown PD. Expression of aquaporin-1 and aquaporin-4 water channels in rat choroid plexus. Biochim Biophys Acta. 2003;1609:80–86. [PubMed] [Google Scholar]
- Speake T, Kajita H, Smith CP, Brown PD. Inward-rectifying anion channels are expressed in the epithelial cells of choroid plexus isolated from ClC-2 ‘knock-out’ mice. J Physiol. 2002;539:385–390. [PMC free article] [PubMed] [Google Scholar]
- Speake T, Kibble JD, Brown PD. Kv1.1 and Kv1.3 channels contribute to the delayed-rectifying K+ conductance in rat choroid plexus epithelial cells. Am J Physiol. 2004;286:C622–C620. [PubMed] [Google Scholar]
- Speake T, Whitwell C, Kajita H, Brown PD. Mechanism of CSF secretion by the choroid plexus. Micros Res Tech. 2001;52:49–59. [PubMed] [Google Scholar]
- Steward MC, Case RM. Principles of ion and water transport across epithelia. In: Davison JS, editor. Gastrointestinal secretion. Wright; London: 1989. pp. 1–31. [Google Scholar]
- Szmydynger-Chodobska J, Chodobski A, Johanson CE. Postnatal developmental changes in blood flow to choroid plexus and cerebral cortex of the rat. Am J Physiol. 1994;266:R1488–R1492. [PubMed] [Google Scholar]
- Venero JL, Vizuete ML, Ilundain AA, Machado A, Echevarria M, Cano J. Detailed localization of aquaporin-4 messenger RNA in the CNS: preferential expression in periventricular organs. Neuroscience. 1999;94:239–250. [PubMed] [Google Scholar]
- Vogh BP, Godman DR, Maren TH. The effect of AlCl3 and other acids on cerebrospinal fluid production: a correction. J Pharmacol Exp Ther. 1987;243:35–39. [PubMed] [Google Scholar]
- Virkki LV, Wilson DA, Vaughan-Jones RD, Boron WF. Functional characterization of human NBC4 as an electrogenic Na+-HCO3- cotransporter (NBCe2) Am J Physiol. 2002;282:C1278–C1289. [PubMed] [Google Scholar]
- Wang CZ, Yano H, Nagashima K, Seino S. The Na+-driven Cl-/HCO3- exchanger: cloning, tissue distribution, and functional characterization. J Biol Chem. 2000;275:35486–35490. [PubMed] [Google Scholar]
- Watts AG, Sanchez-Watts G, Emanuel JR, Levenson R. Cell-specific expression of mRNAs encoding Na+, K+-ATPase alpha- and beta-subunit isoforms within the rat central nervous system. Proc Natl Acad Sci USA. 1991;88:7425–7429. [PMC free article] [PubMed] [Google Scholar]
- Wright EM. Transport processes in the formation of the cerebrospinal fluid. Rev Physiol Pharmacol. 1978;83:1–34. [PubMed] [Google Scholar]
- Wu Q, Delpire E, Hebert SC, Strange K. Functional demonstration of Na+-K+-2Cl- cotransporter activity in isolated, polarized choroid plexus cells. Am J Physiol. 1998;275:C1565–C1572. [PubMed] [Google Scholar]
- Yoshitomi K, Burckhardt BC, Fromter E. Rheogenic sodiumbicarbonate cotransport in the peritubular cell membrane of rat renal proximal tubule. Pflügers Arch. 1985;405:360–366. [PubMed] [Google Scholar]
- Zeuthen T. Cotransport of K+, Cl- and H2O by membrane proteins from choroid plexus epithelium of Necturus maculosus. J Physiol. 1994;478:203–219. [PMC free article] [PubMed] [Google Scholar]
- Zeuthen T, Wright EM. Epithelial potassium transport: tracer and electrophysiological studies in choroid plexus. J Membrane Biol. 1981;60:105–128. [PubMed] [Google Scholar]
- Zlokovic BV, Makic JB, Wang L, McComb JG, McDonough A. Differential expression of Na, K-ATPase α and β subunit isoforms at the blood-brain barrier and the choroid plexus. J Biol Chem. 1993;268:8019–8025. [PubMed] [Google Scholar]